Key considerations for clinical IMS instrumentation
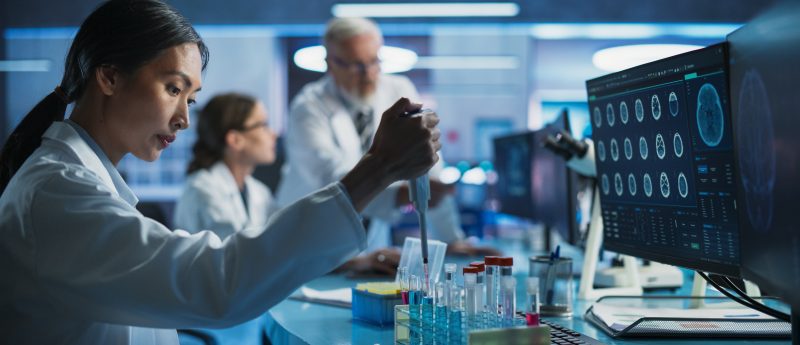
The last two centuries have witnessed enormous strides in health care and disease prevention [1]. Occurrences of many infectious diseases have been mitigated by improvements in sanitation and nutrition, while others have been diminished by the advent of vaccines and antibiotics. For example, Vibrio cholera bacteria initiated global cholera outbreaks throughout the 19th century [1], killing millions of people. In the mid-1800s, John Snow determined contaminated water as the source of transmission [2], and since that time outbreaks have declined. Today, cholera is rare in developed and industrialized regions, although it still remains a threat to areas with unsafe drinking water and poor sanitation. Small pox, an infectious disease once responsible for numerous epidemics and millions of deaths, was virtually eliminated in 1977 due to vaccination campaigns – an unprecedented success attained through enormous efforts [1,3].
The use of statistics to further understand medical conditions and diseases provided significant medical advances linking diseases to external risk factors, such as smoking as a danger for increased development of lung cancer [1,4–7]. Accurate understanding of anatomy and physiology and a foundation for cellular and molecular biology along with innovations in basic research have also led to improved disease detection, screening measures, and treatment strategies [1]. For example, introduction of the Papanicolaou Test (Pap Test) in the mid-1900s to screen for cervical cancer has led to large declines in its incidence and mortality in regions and groups with access to this examination [8–13]. Today, we understand the complexity of many diseases and recognize the potential interaction of environmental and molecular components for those we don’t fully understand.
As a modern medical technology, MALDI IMS facilitates the visualization of in situ molecular distributions and demonstrates great potential as a clinical utility to further the understanding of disease and improve diagnosis, staging, therapeutic decisions and prognosis. However, much work remains to be done in order to validate this technology for applications in the clinical arena. Currently, LC–MS/MS is used routinely for clinical assays, and a review of its advantages and limitations provides insight into desirable features for clinical MALDI IMS instrumentation [14].
High-throughput
Some clinical assays evaluate hundreds to thousands of patient samples per day. As such, a clinical tool used for detection and diagnostics must provide results in a high-throughput manner. Recent advancements in MALDI instrumentation provide lasers that operate as high as 5–10 kHz, producing tissue images in minutes [15–17]. Moreover, these images can contain distributions of thousands of molecular ions, providing an inherent multiplexed analysis with just one scan of the tissue. In another approach, targeted assays can be developed for specific applications and improvements in throughput. For example, quantitation and localization of rifampicin directly from tissue provides equivalent accuracy to that of LC–MS/MS analyses, but yields additional benefits of increased throughput and in situ drug localization [18]. Further, MALDI IMS is amenable to the analysis of tissue microarrays for diagnosis and staging of cancers [19].
Accurate, sensitive and specific
The ability of a diagnostic test or technology to reproducibly report accurate results is of the utmost importance, as these findings directly affect diagnostic and treatment decisions. Sensitivity (the ability of a test to detect the disease when present) and specificity (the ability of a test not to detect the disease when it is not present) are critical. Studies investigating MALDI IMS applications for staging and diagnostic purposes also report high sensitivity and specificity metrics [20,21]. However, as with all clinical assays, specific steps (matrix selection, quantitative accuracy, relevant tissue types, sample storage, etc. ) for each particular application must be extensively validated [14].
Beyond the accuracy of the overall test, mass spectrometry-based assays have the advantage of accurate mass identifications, facilitating the detection of different protein isoforms, the differentiation of modified and unmodified forms, and also the distinction of two different molecules with the same mass and similar chemical composition [22,23]. This is not always feasible with antibody-based assays [24]. Furthermore, MALDI IMS provides spatial resolution of the analytes of interest at a resolution approaching that of bright-field microscopy for H&E staining [25], but with the additional benefit of molecular specificity.
Versatile
A great advantage of mass spectrometry-based technologies is the available variety of workflows. For example, adjustments in sample preparation protocols and analysis methods facilitate the analysis of peptides, proteins, lipids, or small molecules from tissue sections [26]. Methods can be constructed to analyze a broad or narrow range of targets, or only specific targets. Additionally, analyses can be adapted to a variety of tissue types and sizes.
Robust and affordable
Clinical labs often have tens to hundreds of instruments that run assays 24 hours a day, 7 days a week. Thus, for a technology to become clinically viable, it must be robust and affordable. Instruments require regular maintenance and calibration, but extending the length of time that an instrument can perform at top specifications will ease its integration into clinical workflows. For MALDI imaging instrumentation, improved design of the source can reduce tissue accumulation, reducing the cleaning frequency, and expedite cleaning when it is required. Design of more affordable instrumentation that meets the above discussed metrics will facilitate the incorporation of IMS into clinical laboratories. While startup costs may be higher than antibody-based technologies, the versatility and multiplexing achieved by mass spectrometers may justify some of this expense.
To date traditional mass spectrometers have been adapted for imaging purposes. While this has led to many successes, instrument designs specifically targeting imaging applications would facilitate acceptance of the technology at a quicker pace. Newer models are beginning to address advanced capabilities, such as decreased laser spot sizes, higher repetition rate lasers, and well-designed ion sources that decrease cleaning requirements. Additional needs include improved sample stage design to achieve higher spatial resolution. Not only should new mass spectrometers be designed specifically with imaging in mind, but also with enabling clinical utility to facilitate diagnostic use of this powerful technology.
Upcoming feature: Membrane protein preparation strategies
References
- Weatherall D, Greenwood B, Chee HL, Wasi P. Science and technology for disease control: Past, present, and future. (2006). Available from: http://www.ncbi.nlm.nih.gov/books/NBK11740/.
- Snow J. On the Mode of Communication of Cholera. 2nd ed. John Churchill, London, England Available from: https://books.google.com/books?id=-N0_AAAAcAAJ&source=gbs_navlinks_s.
- Fenner F, Henderson DA, Arita I, Jezek Z, Ladnyi ID, World Health Organization. Smallpox and its eradication. World Health Organization, Geneva Available from: http://apps.who.int/iris/handle/10665/39485.
- Doll R, Hill AB. The mortality of doctors in relation to their smoking habits; A preliminary report. Br. Med. J. 1(4877), 1451–1455 (1954).
- Doll R, Hill AB. Mortality in relation to smoking: Ten years’ observations of British doctors. Br. Med. J. 1(5396), 1460–1467 (1964).
- Wynder EL, Graham EA. Tobacco smoking as a possible etiologic factor in bronchiogenic carcinoma; A study of 684 proved cases. J. Am. Med. Assoc. 143(4), 329–336 (1950).
- Bayne-Jones S, Burdette WJ, Cochran WG, et al. Smoking and Health. U.S. Department of Health, Education, and Welfare, Washington D.C Available from: http://profiles.nlm.nih.gov/NN/B/B/M/Q/.
- Cannistra SA, Niloff JM. Cancer of the uterine cervix. N. Engl. J. Med. 334(16), 1030–1037 (1996).
- Clarke EA, Anderson T. Does screening by “Pap” smears help prevent cervical cancer? The Lancet. 314(8132), 1–4 (1979).
- Cramer DW. The role of cervical cytology in the declining morbidity and mortality of cervical cancer. Cancer. 34(6), 2018–2027 (1974).
- Koss LG. The Papanicolaou test for cervical cancer detection: A triumph and a tragedy. J. Am. Med. Assoc. 261(5), 737–743 (1989).
- Quinn M, Babb P, Jones J, Allen E. Effect of screening on incidence of and mortality from cancer of cervix in England: evaluation based on routinely collected statistics. Br. Med. J. 318(7188), 904 (1999).
- Viswanathan A, Dizon DS, Gien LT, Koh W-J. Cervical Cancer. In: Clinical Radiation Oncology, Fourth Edition. Gunderson LL, Tepper JE (Eds.). Elsevier, Philidelphia, PA, 1173–1202 (2016) [cited 2016 Apr 13]. Available from: https://www-clinicalkey-com.proxy.library.vanderbilt.edu/#!/content/book/3-s2.0-B9780323240987000587?scrollTo=%23hl0000599.
- van den Ouweland JMW, Kema IP. The role of liquid chromatography-tandem mass spectrometry in the clinical laboratory. J. Chromatogr. B Analyt. Technol. Biomed. Life. Sci. 883-884, 18–32 (2012).
- Spraggins JM, Caprioli RM. High-speed MALDI-TOF imaging mass spectrometry: Rapid ion image acquisition and considerations for next generation instrumentation. J. Am. Soc. Mass Spectrom. 22(6), 1022–1031 (2011).
- Prentice BM, Chumbley CW, Caprioli RM. High-speed MALDI MS/MS imaging mass spectrometry using continuous raster sampling. J. Mass Spectrom. 50(4), 703–710 (2015).
- Ogrinc Potočnik N, Porta T, Becker M, Heeren RMA, Ellis SR. Use of advantageous, volatile matrices enabled by next-generation high-speed matrix-assisted laser desorption/ionization time-of-flight imaging employing a scanning laser beam. Rapid Commun. Mass Spectrom. 29(23), 2195–2203 (2015).
- Chumbley CW, Reyzer ML, Allen JL, et al. Absolute quantitative MALDI imaging mass spectrometry: A case of rifampicin in liver tissues. Anal. Chem. 88(4), 2392–2398 (2016).
- Groseclose MR, Massion PP, Chaurand P, Caprioli RM. High-throughput proteomic analysis of formalin-fixed paraffin-embedded tissue microarrays using MALDI imaging mass spectrometry. Proteomics. 8(18), 3715–3724 (2008).
- Yanagisawa K, Shyr Y, Xu BJ, et al. Proteomic patterns of tumour subsets in non-small-cell lung cancer. Lancet. 362(9382), 433–439 (2003).
- Rauser S, Marquardt C, Balluff B, et al. Classification of HER2 receptor status in breast cancer tissues by MALDI imaging mass spectrometry. J. Proteome Res. 9(4), 1854–1863 (2010).
- Wenke JL, Rose KL, Spraggins JM, Schey KL. MALDI imaging mass spectrometry spatially maps age-related deamidation and truncation of human lens squaporin-0. Invest. Ophthalmol. Vis. Sci. 56(12), 7398–7405 (2015).
- Khatib-Shahidi S, Andersson M, Herman JL, Gillespie TA, Caprioli RM. Direct molecular analysis of whole-body animal tissue sections by imaging MALDI mass spectrometry. Anal. Chem. 78(18), 6448–6456 (2006).
- Alomari AK, Klump V, Neumeister V, Ariyan S, Narayan D, Lazova R. Comparison of the expression of vimentin and actin in spitz nevi and spitzoid malignant melanomas. Am. J. Dermatopathol. 37(1), 46–51 (2015).
- Spraggins JM, Rizzo DG, Moore JL, Noto MJ, Skaar EP, Caprioli RM. Next-generation technologies for spatial proteomics: Integrating ultra-high speed MALDI-TOF and high mass resolution MALDI FTICR imaging mass spectrometry for protein analysis. Proteomics. (2016).
- Anderson DMG, Spraggins JM, Rose KL, Schey KL. High spatial resolution imaging mass spectrometry of human optic nerve lipids and proteins. J. Am. Soc. Mass Spectrom. 26(6), 940–947 (2015).