Analytical considerations in peptide and protein bioanalysis
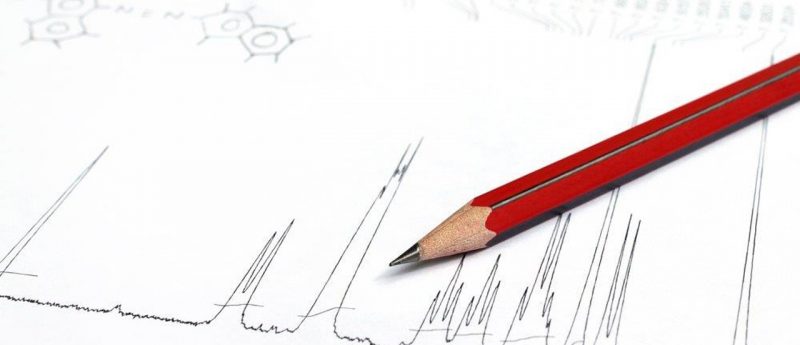
For many bioanalytical scientists today, the transition from traditional ‛small molecule’ quantification to the world of peptides and proteins continues to be difficult. Some of the greatest challenges include: adapting to new techniques, longer more complex multi-stage workflows, understanding handling differences, and the simple fact that achieving the required bioanalytical sensitivity and specificity for large molecules is a greater task than for small molecules. Complicating these inherent challenges further is the increasing complexity in the nature of candidate therapeutics being explored as we move from peptides to proteins and the current generation of biopharmaceuticals, such as human monoclonal antibodies and antibody drug conjugates (ADCs). The following analytical considerations, segmented into discussions around liquid chromatography (LC), mass spectrometry (MS), and sample preparation, represent the culmination of our own learning and experiences as well as that of the broader research industry. The guidelines put forth are relevant not only to therapeutic and endogenous peptide quantification, but also to the quantification of large therapeutic and endogenous proteins (>10kDa) via the widely accepted surrogate peptide approach.
In terms of LC, for most peptides, traditional fully porous C18 stationary phases will provide optimal performance for the majority of separations. However, the more recent introduction of superficially porous particles may provide higher peak capacity, and thus reduce the risk of co-elutions. For larger (> ~2000 Da) peptides in particular, sub 2µm particles, larger chromatographic pores (i.e. 300Å), shorter ligands, and the aforementioned superficially porous particles may all provide additional improvements in resolution, peak width, and reduced run times. Rising in popularity is also the use of charged-surface stationary phases, which can reduce secondary interactions, and in doing so, provide further peak shape improvements. From an optimization and troubleshooting perspective, decreasing flow rates to the 200–400 µL/min range, elevating temperature to 60°C, and because ballistic gradients are NOT recommended for large molecule separations (as precipitation may occur), shallowing the gradient slope may all reduce carryover, improve peak shape, and increase sensitivity for peptide quantification.
Perhaps one of the most analytically challenging and less predictable components of large molecule methods is non-specific binding. Peptides and proteins tend to bind to many surfaces (vials, plates, LC tubing, column stationary phases, injectors, etc.) in a concentration dependent, yet non-linear manner. While the use of ‛low binding’ consumables offers some protection, none eliminate the problem completely. The best practice is to combine a low binding consumable with the addition of a carrier protein to the sample, and the correct percentage of organic and modifier (often acid). The ideal percentage of organic depends on the peptide, but in order to obtain and maintain full solubility, as much as 30–40% organic, for particularly large and/or hydrophobic peptides such as amyloid beta peptides or insulins, may be required.
Acid content can be thought of in a similar manner. While most peptides need only 0.2–1% formic or trifluoroacetic acid for solubility, as much as 10–30% may be necessary for the larger, stickier peptides. Another trick that has proven effective for both reducing carryover and improving solubility during LC analysis or SPE is the incorporation of trifluoroethanol (TFE) into mobile phase B and/or in the SPE elution solvent. One final analytical note on chromatography is that it is common to see improved recovery, peak shape and reproducibility if the chromatographic system and column are passivated prior to use. This can be accomplished in a number of ways such as performing 10–30 large volume injections of precipitated plasma or a highly concentrated (mg/mL) protein solution.
With regards to MS, application of several key recommendations can significantly increase the specificity, sensitivity and reliability of MS detection. Specificity is valuable not only in its own right, but also as it relates to sensitivity, as the two are invariably interrelated. The better the specificity, the greater the signal-to-noise (S:N), and the better the sensitivity. Avoiding immonium ions, water losses, and adducts may seem obvious, as the latter two also relate to small molecule analysis. What is not as apparent, however, is the critical importance of choosing the highest m/z precursor and fragments possible, yet balancing that choice with absolute sensitivity requirements. In particular, we tend to see greater specificity and related sensitivity when using precursors and fragments with m/z values greater than ~800–1000. When choosing a tandem quadrupole system, one should consider a system with a mass range of ~2000 amu on both quadrupoles to allow for selection of these higher m/z precursors and fragments, thus enabling the highest potential sensitivity.
The relationship between specificity and sensitivity is a complicated one. One must be aware that highly intense fragments that can be as much as an order of magnitude greater in sensitivity than other options, can actually completely lack specificity. Common culprits are the aforementioned immonium ions, water losses, and adducts. It is generally good practice to choose b or y sequence ions, with those more internal to the sequence often affording greater specificity than small N- or C-terminal fragments. B and y ions are produced through sequential cleavage at the amide bonds in between individual amino acids. This results in a very predictable series of fragments in positive ion electrospray (in silico digestion models are very effective at this prediction). In contrast, collision induced dissociation (CID) in negative ion mode may produce high intensity fragments, but it is poorly understood and often results in spectra with high background and poor analyte S:N due to lack of specificity.
Unlike small molecules with precursor charge states of +/- 1, LC flow rate can dramatically influence the nature and relative abundance of specific multiply charged peptide precursors. Therefore, one must always tune peptides at the chromatographic flow rate that will be used during sample analysis. This is achieved effectively by tee-ing the analyte stream into the LC effluent. A reasonable mobile phase composition for tuning would be ~30% organic, as it sits in the middle of the organic range where peptides tend to elute and is a good surrogate if a single analytical condition must be chosen for tuning. Finally, monitoring of several MRM transitions during method development is important to ensure that the most specific MRM is identified and to monitor and account for any possible changes in charge state distribution observed with changes in concentration.
The final topics that warrant careful consideration are sample preparation and the related topic of peptide handling. While protein precipitation (PPT) is often used in discovery studies and/or where low detection limits are not required, it fails to provide the specificity required for low-level detection and pg/mL sensitivity. As the peptide of interest increases in size, the utility of PPT is further reduced. It can, however, be employed very successfully as a cheap and easy method of pre-fractionating a sample prior to SPE or other techniques. For smaller peptides (up to approximately 2000 Da), a ratio of 2:1 or 3:1 organic to plasma will usually result in high peptide recovery. Due to solubility limits, for larger peptides (above 3000 Da or so), a ratio of 1:1 successfully precipitates unwanted high abundance proteins while maintaining solubility of the peptides of interest. Not surprisingly, SPE remains one of the simplest, most effective ways to purify therapeutic, endogenous, or tryptic peptides. While many employ straight reversed-phase sorbents, greater specificity is obtained when mixed-mode chemistries (sorbents enabled with retention via either reversed-phase or ion-exchange) are applied. When peptides are bound to the ion-exchange moiety of a mixed-mode sorbent, the SPE builds orthogonality into the assay as a whole. This is often one of the single most powerful techniques for increasing overall method specificity. A few simple guidelines, primarily related to solubility considerations, increase the effectiveness of mixed-mode sorbents. For example, although the organic composition of the eluate should not exceed 75%, increasing the percentage of acid/base in an elution solution can improve peptide recovery, especially for larger or more hydrophobic peptides. Knowing the isoelectric point (pI) of the peptide of interest not only helps to predict whether to use anion or cation exchange SPE, but it can also guide choices to improve solubility. Peptides with a basic pI are more soluble in acidic solutions, while the converse is true for peptides with acidic pIs. Similarly, predictive software can provide a preview to those peptides which are likely to exhibit protein binding, non-specific binding, and/or problems with solubility. Generally speaking, one should avoid evaporation of extracts containing the desired peptides or proteins, as this can result in partial or complete losses due to adsorption to surfaces. This can be mitigated through the use of carrier protein as previously mentioned. Finally, when TFE is added to the final eluate, it can improve SPE recovery by improving peptide solubility.
Bioanalysts continue to face new and more challenging obstacles each year. As the complexity of biotherapeutics continues to evolve, so must the level of our understanding and the tools at our disposal.